
The Connected Connectome
It’s usually older professors who tell tales about how things were different back when they started out. Asa Barth-Maron isn’t done with his Ph.D., but his early grad school days already feel like ancient history.
Barth-Maron works in Rachel Wilson’s lab at Harvard University studying interneurons in the fruit fly antennal lobe, a brain region that processes olfactory information gathered by sensory neurons in the flies’ antennae before relaying it to higher brain centers. When he joined Wilson’s lab in 2015, the basic approach to studying a neuron’s function was to record from it in vivo, see what stimuli it responded to or how its activity correlated with behavior, and then try to determine how the neuron was embedded in a circuit.
Light-microscopy data gave hints of that circuit, but not a complete picture — it was akin to a subway map that leaves out the transfer points. Determining whether adjacent neurons were actually connected required laborious physiological investigations, like having to ride random trains to see which lines connected. “We would essentially take shots in the dark,” Barth-Maron says.
Fast forward six years, and researchers have a precise neuronal map at their fingertips. “Now you can pull up a website,” he says, “search for your cell of interest, and see what inputs and outputs it receives and sends.”
This open-access resource is the fruit of the FlyEM connectomics project based at the Howard Hughes Medical Institute’s Janelia Research Campus, which uses electron microscopy (EM) and state-of-the-art reconstruction technologies to map the complete Drosophila brain at the level of individual synapses. In September 2020, the project yielded a landmark 108-author paper in eLife describing the structure of roughly 25,000 neurons in the fly’s central brain — the so-called hemibrain — and their 20 million connections.
Connectomics is so profoundly changing Drosophila neuroscience that Larry Abbott, a theoretical neuroscientist at Columbia University, says the field can now be divided into two epochs: B.C. and A.C. — Before Connectome and After Connectome. “For the people cracking the circuits, I don’t think that’s an exaggeration,” says Abbott, who is also an investigator with the Simons Collaboration on the Global Brain. “Everybody is pretty much consulting it all the time now.”
In August 2020, Abbott and 23 other experts published a whopping 192-page preprint on the bioRxiv that explored the implications of the hemibrain data for mushroom-body neurobiology. The mushroom body is an olfactory processing area downstream of the antennal lobe that has long been regarded as the fly’s olfactory learning center. It has been intensely studied, and yet the FlyEM data revealed many unexpected things about it.
“The biggest surprise was the existence of a specific visual pathway that stays segregated and seems to have a special status,” Abbott says. Previous work had hinted that the mushroom body is involved in crude visual functions, such as avoiding large obvious stimuli. But knowing precisely which visual pathways that enter, exit and operate within it will help modelers unpick how vision affects the mushroom body.
The preprint examines how the new connectivity data fit with existing physiological, molecular and behavioral data regarding the mushroom body — and with theoretical models of the region’s function. Abbott believes it is this cross-fertilization of multiple rapidly evolving disciplines that makes Drosophila neurobiology so exciting right now — the emerging connectome is facilitating the integration of different approaches.
By doing so, it’s helping theorists build more biologically realistic models and allowing physiologists to more incisively explain the neural activity they observe. Researchers also believe that invertebrate connectomics holds many lessons for emerging mammalian connectome projects — including lessons in the alternative ways connectomes can be generated.
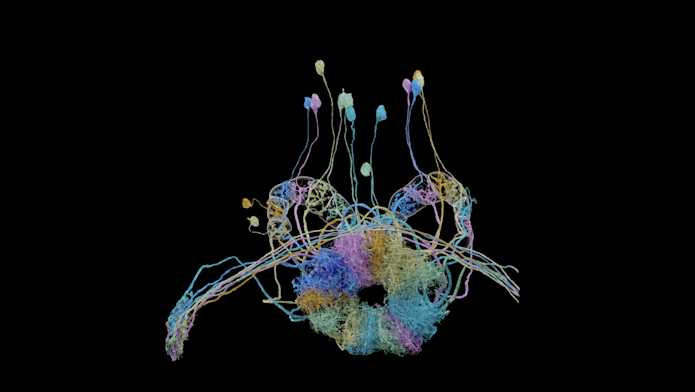
By clicking to watch this video, you agree to our privacy policy.
From a worm to a fly
The audacious idea of mapping a complete nervous system using EM originated in the mid-1960s. Wanting to study brain function by observing how genetic mutations affected both behavior and neuronal structure, the biologist Sydney Brenner sought a new model organism with a nervous system small enough to be mapped in its entirety. He chose the nematode Caenorhabditis elegans and, in 1969, recruited the physicist John White to lead a mapping project.
White worked with two technicians: Nicol Thomson sectioned and imaged tissue, while Eileen Southgate printed out Thomson’s micrographs on 12-by-16-inch pieces of paper, overlaid transparent plastic, and traced every neuron and synapse in colored pens. In 1984, after 15 years of work, the foursome had described 302 neurons.
Their 340-page paper was a neuroscience milestone, contributing to Brenner’s 2002 Nobel Prize and providing a connectome that’s been an invaluable reference work for C. elegans neurobiologists ever since.
But some researchers think it was so ahead of its time that its value took decades to be fully realized. “The worm had a complex legacy,” says Viren Jain, who works on connectomics at Google and collaborates with the FlyEM team. When the wiring diagram was published, next to nothing was known about the physiology of nematode neurons, and behavioral studies of the animal were rudimentary.
After its publication, EM analysis remained a relatively obscure neuroscience specialism because it was so time-consuming. Each of its main stages — tissue sectioning, preparing and imaging those sections, then analyzing and reconstructing the images to create a three-dimensional map — was a laborious process. And every step demanded precision. Connectomics has no room for error: Losing a single tissue section can derail reconstruction of an entire nervous system because often the processes passing through that plane can no longer be confidently traced.
In the mid-2000s, however, Winfried Denk and collaborators, then at Heidelberg’s Max Planck Institute for Medical Research, automated sectioning and imaging. Around the same time, Jain, working with Srinivas Turaga in Sebastian Seung’s lab at the Massachusetts Institute of Technology, started developing ways to automate image analysis and stitch together two-dimensional images of individual EM slices into three-dimensional structures. They used computer vision techniques called convolutional neural networks (CNNs). Today, CNNs are fundamental to most computer vision work, but the technique was a surprising choice at the time, Jain says. The group’s successes — which included helping Denk reconstruct a mouse retina — made CNNs a central component of connectomics.
The two key reconstruction steps that researchers have automated using artificial intelligence are aligning sections, so that elements of each micrograph match the corresponding points on micrographs of neighboring sections, and segmentation, whereby segments of cells are rendered as distinct 3D volumes that can be linked together to generate a neuron’s morphology. In addition, algorithms derived from machine learning have been developed to recognize synapses.
The emergence of these various technologies made a connectome of an entire 100,000-neuron Drosophila brain seem like a feasible proposition. Unlike the researchers working with the C. elegans connectome, fruit fly neurobiologists, Jain says, “had questions they wanted to answer using that data.”
The first group to image an entire Drosophila brain was led by Davi Bock, then at Janelia, and used transmission EM (TEM). In 2018, this project, known as full adult fly brain (FAFB), published its data, making them freely available for anyone to use. The downside was that the images still required much analysis and reconstruction — at that point, mainly by hand.
In contrast, the FlyEM project, led by Gerald Rubin, Louis Scheffer and Stephen Plaza, used higher-resolution — but slower and costlier — focused ion beam scanning EM because the clearer raw data would enable them to use automated analytical techniques. The CNN algorithms rapidly generated a first draft of the hemibrain. But even so, the algorithms were not foolproof, meaning the draft had to be manually proofread — which took two calendar years, or an estimated 50 person-years.
The result is the neuPrint website, which Barth-Maron lauded. It now has more than 1,700 registered users who can pick out an individual neuron and immediately view the cell’s structure and a list of all its synaptic partners.
There is, however, a twist. While the FlyEM team is preparing to reconstruct a whole brain, Seung, now at Princeton University — with Mala Murthy, a Drosophila neurobiologist also at Princeton — has launched a project to analyze the FAFB dataset. Advances in machine learning made by Seung’s group allowed them to assemble a draft connectome from the TEM micrographs — meaning a draft of a whole brain.
Seung and Murthy also developed new proofreading software and deployed it using another tactic that Seung has helped foster: crowdsourced proofreading
To show me how this works, project manager Claire McKellar gave me a tour of the FlyWire website, where the connectome resides. She pulled up two neurons at random, one of which apparently had two cell bodies (an obvious case of segments being erroneously merged by the algorithms), whereas the dendritic tree of the second was clearly truncated. As I watched, McKellar found the second neuron’s missing dendrites in the adjacent section and attached them, as the system automatically logged the update and who had made it.
FlyWire currently has roughly 100 active users, and, McKellar says, around 4,000 neurons have so far been reconstructed. Murthy says that most current users are neuroscientists “motivated to do the proofreading in their circuit of interest” — particularly if that circuit isn’t within the hemibrain volume or if it has important connections beyond that volume. Additionally, the project is also recruiting full-time proofreaders to cover terra incognita. “Our goal,” says Murthy, “is to achieve a whole-brain connectome within the coming year.”
She acknowledges the “friendly rivalry” that exists between the projects but stresses the constant sharing of expertise and resources. “It isn’t clear at this moment,” she says, “where the first whole-brain connectome will come from. Whether it’ll be primarily through advances on the algorithmic side or on the data-collection side.”

From micrographs to cell types and variability
Reconstructing the neurons is, however, just the first step. Next, each one must be labeled and classified, so that ultimately the circuitry and wider organization of the fly brain can be resolved. “In the genome you analyze it by gene,” says Marta Costa, of the Drosophila connectomics group at the University of Cambridge; “in the connectome you analyze it by cell type.”
Previous research, which defined Drosophila neurons by the genes they express and constructed a vast library of transgenic flies expressing GFP in select neurons, estimated that fruit flies possess 5,000 to 10,000 distinct types of neurons — some of which consist of only a single left-right pair of cells. These transgenic flies allowed the researchers to build a catalog of the morphologies of genetically identified neurons using light microscopy. Algorithms that find these morphologies in EM data have helped semi-automate the identification of neuronal types, says Costa. But the neurons’ EM-derived structure and connectivity indicated even more types. The FlyEM team identified about 5,600 in the hemibrain’s roughly 25,000 neurons, about half of them new. “We’re still seeing things that we’ve never seen before,” Costa says.
Often, the specificity of individual cell types was unanticipated. Abbott highlights, for example, how the mushroom body’s well-studied population of dopamine neurons turned out to be composed of multiple different types. Their unique properties suggest they each serve discrete functions.
But there are still questions about how much the structure and connectivity of a given type varies from brain to brain, which will require comparing multiple connectomes to resolve. This is something C. elegans scientists have begun to do. Mei Zhen, a neuroscientist and EM specialist at the Lunenfeld-Tanenbaum Research Institute and the University of Toronto, recently led a study reconstructing eight C. elegans connectomes from different developmental stages. The work aimed to uncover key features of development; the team found, for example, that sensory and motor systems are progressively remodeled, but decision-making interneuron hubs remain stable over time. But the study also showed that connectivity varies significantly between animals even in a nervous system considered to be very much hard-wired. Roughly 40 percent of synapses in adults, mainly between weakly connected pairs of neurons, didn’t appear in all worms.
To further probe variability and its causes, Zhen and her collaborators are starting experiments using connectome-wide EM in which nematodes will be reared in different conditions to determine how environmental challenges affect neuronal development. “We want to know how certain inputs shift or even generate a new set of connections that we do not see at baseline,” she says.
Studies such as these will help researchers determine the extent to which fly, worm and other brains are hard-wired. This information alone has very important implications for future circuit models. Many existing computational models rely heavily on activity-dependent wiring rules to shape connectivity and function; the degree to which connections between specific neurons are hard-wired or plastic will indicate the extent to which such rules can realistically be used.

From cells to circuits and models
When it comes to using connectomics data to understand brain function, users can be broadly split between physiologists and theorists, although the two camps increasingly overlap.
Even though the Wilson lab researched antennal-lobe neurophysiology extensively for years, Barth-Maron says that EM data have unearthed many unexpected connections. These include findings that different interneuron subpopulations are under distinct neuromodulatory regulation and that not all local inhibitory interneurons there inhibit the incoming sensory neurons; one curious subpopulation innervates mainly one another. Such observations present novel ideas that are then investigated physiologically.
In Murthy’s lab, connectivity data from the FlyWire project have so far been vital to two projects. In one, postdoc Christa Baker recorded from various neurons in the auditory pathway that were identified using transgenic fly strains. She charted activity in response to the fly’s two types of courtship song, finding that the two song types were encoded by surprisingly overlapping populations of neurons.
“This immediately led to the next question: What’s the organization of this pathway?” Murthy says. “Who connects to who? How is information transmitted throughout the pathway?”
Baker spent roughly a month finding the respective neurons in the draft connectome and tracing their connections. From there, the group could generate a new model of auditory processing. “It really changed our view of how auditory processing works,” Murthy says.
Theorists are also enjoying using the connectome to develop models that more accurately reflect biology. One widely heralded example of how connectivity data are confirming previous theoretical work involves the fly’s central complex and its function in navigation.
Vivek Jayaraman and colleagues at Janelia established a virtual reality system to record neural activity as flies oriented themselves on a small floating ball in response to visual cues. Building on older work concerning mouse navigation, Jayaraman’s group developed a model of a fly’s internal compass system where neural activity moved around a ringlike structure according to how far the fly had turned.
Turaga, now a group leader at Janelia, says that when the EM data came in, “a beautiful crystalline architecture proposed by theory was there in the connectome.” (For more, see “In Fruit Fly Brain, a Ring for Navigation.”)
Abbott is in awe of how well the project turned out. The model had incorporated a sinusoidal pattern of neuronal connectivity. Functions based on sine waves are powerful mathematical tools, but they’d perhaps always been viewed as something of a useful conceit. But in the connectomics data, the synaptic distribution was indeed sinusoidal. “This crazy assumption that the theorists probably made just to make it easy matches perfectly,” Abbott says. “It’s the theorist’s dream come true.”
Theorists are also now able to use connectomics data as the starting point for building new models. In 2018, Turaga and colleagues posted a preprint describing a simple model of early visual processing based on synaptic connections and weights derived from EM data. This model worked better than one based initially on random connections.
Importantly, such models include another feature: Because the artificial neurons now correspond to real-life neurons, the activity of the artificial neurons can be cross-checked against recorded activity from real neurons. “If you didn’t have this connectome, then any neuron can perform any role at all,” Turaga says.
In his 2018 model, the activity of certain individual neurons matched the activity of corresponding neurons in flies — but it didn’t match for all of them. Turaga is now building a richer model incorporating more connectivity data, which performs even better than the original.
He cautions, however, that connectivity does not tell modelers everything they need to know to constrain their models. One of the most powerful things a connectome can do is say that two neurons are not connected, because that definitively rules out using such a connection in a model. However, the physiological properties of most neurons and synapses remain unknown, and these parameters strongly influence a model’s performance. “In terms of what can happen, the space is still large,” he says. “We’re learning the limits of what we can and cannot interpret from electron microscopic images.”
One recent advance that helps constrain models has been the development of machine learning techniques that can phenotype synapses. Previously, owing to their overwhelming structural similarity, EM images from Drosophila couldn’t indicate if a synapse was inhibitory or excitatory —leaving models free to use either sign. The new algorithm can distinguish between synapses that are glutamatergic, GABAergic or cholinergic.
“Modeling is all about constraints,” says Abbott. He’s enjoying how the After Connectome era is holding modelers to higher standards. If theorists build models unconstrained by biological data, he says, “we can think of a zillion ways to get the job done. We want to get the right one — the one that the fly’s using.”
—
The Connected Connectome Part 2: Beyond the Fly
Success in flies and worms has inspired several efforts to reconstruct more complex mammalian brain circuits. Reconstructing a whole mouse brain using electron microscopy is the next logical step, but scaling from a fly to a mouse brain presents serious challenges.