
Karin Öberg’s Search for the Chemical Basis of Planet Formation
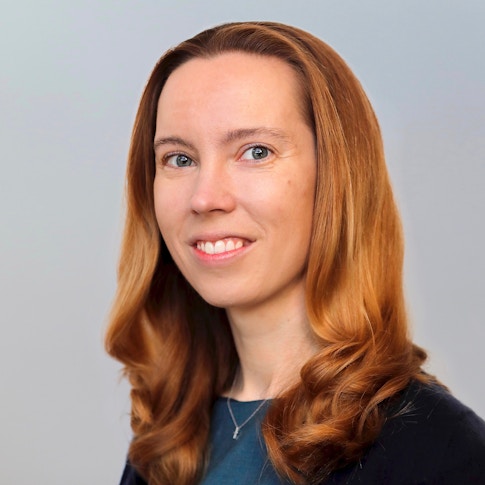
It could be said that Earth’s most consequential attribute is its chemistry. After all, if our rocky little orb sitting 93 million miles away from its star had been born with even a slightly different molecular makeup, we very likely would not be here.
Despite these long odds for life, rocky planets like ours are not overly rare in the universe. Astronomers have detected thousands of exoplanets and suspect that almost every star in our galaxy has at least one planet in its orbit. Although some of these planets can be classified as gas giants like Jupiter, a sizeable number of them appear at first glance to be like Earth in many ways, and to orbit in the so-called habitable zone: the ‘just right’ distance from their star to yield liquid water, a known prerequisite for life.
“If you have a planet that’s kind of like Earth, how often do you then get the chemical environment that we think is conducive to the origins of life?” says Karin Öberg, an astrochemist at Harvard University. Öberg is also a Simons Investigator in Astrophysics and an investigator with the Simons Collaboration on the Origins of Life.
That is one of the burning questions that motivate Öberg and others like her. And as tools for observing the cosmos have advanced in recent years, she and her colleagues are poised to make big strides toward answering it.
In the early days of astrochemistry, researchers were relegated to studying the interstellar medium, the exceedingly cold and vast expanse of rarefied gas and dust between star systems, on large scales. Today — most notably with the help of the Atacama Large Millimeter/submillimeter Array (ALMA), which began collecting data in 2011 — they can zoom in on the regions of space where stars and planets form. “It’s like Google Earth, where the first versions were one big blob and now you can see every street and house in the city,” says Ewine van Dishoeck, an astrochemist at Leiden University and president of the International Astronomical Union.
Recently, researchers began to glimpse in detail the composition of molecules within protoplanetary disks, cosmic structures of swirling gas and dust that spin around young stars and give birth to new planets. The researchers inform their cosmic observations with results from both laboratory experiments and computer simulations, which enable them to probe the physical forces and chemical reactions that might explain how these molecules are produced and how they interact. Altogether, with these data, “we are finally starting to see what happens at the molecular level as planets are being born,” says Edwin Bergin, an astrochemist at the University of Michigan.
As Öberg envisions it, by understanding the distribution of water, carbon, oxygen and nitrogen within a given protoplanetary disk, researchers should one day be able to predict the features of planets forming there — including whether they contain the ingredients for emergent life. But much remains to be discovered before such a “predictive theory of planet formation” can be achieved, she says. “We are in an era where we have a basic qualitative framework that provides a reasonable explanation for the chemistry we observe and what it might mean for planet formation, but now we have to move to a quantitative version.”
Intergalactic chemistry
Here on Earth, molecules faithfully observe the laws of thermodynamics. But chemistry in space works differently, mostly because of density. On our planet, a square centimeter of air contains thousands of trillions of molecules. In star-forming and planet-forming cosmic regions, that number dwindles by many, many orders of magnitude. But the interstellar medium is the most sparsely populated of all: A square centimeter of space might house but a single molecule. This rarefied environment sets up special conditions for molecules in the gas phase — which, in space, is 99% of them. On our crowded planet, gas molecules collide frequently, and the energy produced when two molecules form a bond can easily be carried away by a third molecule that bumps into them. Not so in space, Öberg explains. “That really limits the kinds of reactions that can occur there.”
Because of these limitations, much of the key chemistry relating to planet formation takes place in the solid state, on the icy surface of submicron silicate particles or grains, in protoplanetary disks. Inside these disks, water and organic molecules form through different pathways than they do on Earth: Individual atoms freeze out onto the grains, essentially wiggling around until they find each other. “This kind of ice chemistry offers pathways to chemical complexity in space that would otherwise be impossible,” Öberg says.
These grains are the seeds of planet formation, a drawn-out event that takes millions of years. This expansive timescale creates a thorny challenge for scientists who seek to understand it. “Astronomy is different from biology or planetary chemistry in that by and large, when you’re studying a process, you don’t get to see the process happening,” says Eric Herbst, an astrochemist at the University of Virginia. “You see different objects in the sky, and you put together a story as to the sequence of events.”
For planet formation, that sequence begins in the protoplanetary disk, where icy grains collide and grow into pebbles. These pebbles concentrate in the disk’s mid-plane, where their mutual gravitational attraction creates distinct pebble pileups. These pileups are the building blocks of planets. They continue to grow by gravitationally attracting more pebbles, as well as larger collections of grains called boulders. Once they reach planet size, their gravitational pull becomes strong enough to hold on to the gas from the disk, forming the beginnings of an atmosphere. “We think all planets, whether they become Jupiters or Earths, start forming this same way,” Öberg says.
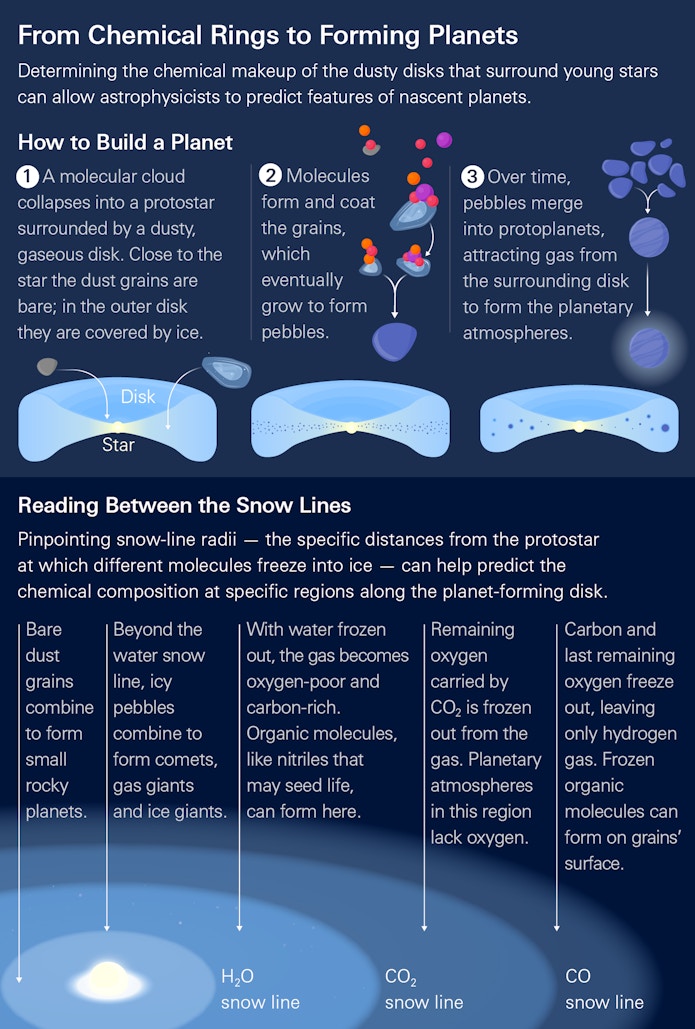
Theoretical roots
Many planets can be born from a single protoplanetary disk. Ultimately, though, where in the disk a planet forms plays a major role in determining which type of planet it will become. One defining characteristic of a planet’s atmosphere is its carbon-to-oxygen ratio, as this regulates much of the subsequent chemistry. This atmospheric ratio is set by the amount of oxygen and carbon a planet accretes for the disk. Importantly, this ratio is different at different locations on the disk. This is mainly because the temperature at a given location in the disk determines what chemistry is possible, including how much carbon and oxygen can be in the gas phase. A foundational paper that Öberg co-authored in 2011 established the conceptual framework for the chemistry underpinning this notion. The idea emerged at the end of her graduate school days at the Leiden Observatory, Öberg says, but it seemed so obvious to her that she filed it away until almost two years later, when she mentioned it almost in passing to fellow astrochemist Bergin, as well as theorist Ruth Murray-Clay (now at the University of California, Santa Cruz), who both urged her to write it up immediately.
The underlying concept is simple. It’s hotter toward a protoplanetary disk’s center, and colder at its outskirts. The three main carbon and oxygen molecules swirling around the disk— H20, CO, CO2 — sublimate at different temperatures and therefore at different distances from the disk’s center. Water freezes close to the center; carbon monoxide freezes further out, while maintaining its gaseous state closer to the center. Carbon dioxide freezes somewhere in between. Why does this matter for the carbon and oxygen gas ratio? Both water and carbon dioxide contain more oxygen than carbon. Öberg’s 2011 paper postulated that any gas located toward the center of the disk would therefore be oxygen-rich, while gas in outer disk regions — where only carbon monoxide is still in the gas phase — would have the same amount of carbon and oxygen. Where exactly the transition from gas to ice happens for any of these molecules would depend on how hot or cold the disk is. For example, in the disk that formed our solar system, researchers think that water froze at about 3 astronomical units (AU) from the center, with carbon monoxide freezing at about 20 AU. (In astronomy, 1 AU is defined as 93 million miles, or the distance between the Earth and the sun.)
Astrochemists have known for decades that the ratio of carbon to oxygen in gas is a fundamental parameter of a star system’s chemistry, says Klaus Pontoppidan, an astronomer at the Space Telescope Science Institute in Baltimore, Maryland. “But it was always applied to different systems, like evolved stars or interstellar medium — not planet formation.”
The 2011 paper, co-authored by Öberg, Bergin and Murray-Clay, wasn’t just a landmark for astrochemists studying planet formation. It quickly became a much-needed bridge between astrochemistry and exoplanet research. It is now highly cited by researchers in both fields because theoretically, it directly ties a planet’s chemical origin story to its ultimate molecular composition. “Now that people are measuring atmospheric composition and carbon-to-oxygen ratios on exoplanets, it was really exciting that this might be a way to track back the history of how these planets formed,” says Ilse Cleeves, an astrochemist at the University of Virginia.
From theory to practice
It’s not yet clear how precisely the theory will play out in practice. At present, astrochemists can’t directly measure how the temperature changes along a disk’s radius to predict where molecules might shift from gas to ice. But data from the ALMA telescope is yielding some promising insights. ALMA is an interferometer, which can detect radio wavelengths emitted by many small molecules in a disk’s gas phase. It captures images of each wavelength at a spatial resolution of about 10 AU, making it at least an order of magnitude better than other telescopes of its type.
To put that in perspective, our solar system has a radius of more than 100 AU. But all eight planets in our solar system sit within 30 AU of the sun. In most protoplanetary disks, planets are thought to form within 50 or so AU of their star. “The planet-forming region in disks is within 50 AU, and the interesting planet-forming region is within 10-20 AU, so it’s a huge step forward,” says Catherine Walsh, an astrochemist at the University of Leeds.
This means that while previous telescopes could capture the overall quantity of different molecules in the disk, they could not map how those molecules were distributed in the regions most related to planet formation, says Öberg. One of the first discoveries she and her collaborators made with ALMA was that carbon monoxide seems to be disappearing from the pool of gas — and thus, presumably, freezing out onto grains — at around 30 AU. The observation was “so rewarding,” she says, because “it was one of the first times we knew we had seen a very concrete change in the chemical environment of a disk.” This also provided the first observational anchor for the carbon-to-oxygen ratio theory, since it relies on precise understanding of where in disks different molecules — including carbon monoxide — freeze out.
A goal for future ALMA studies is to locate exactly where in disks water freezes out; during the formation of our solar system this happened at around 3 AU. Whether a planet forms inside or outside this zone where water freezes — called the snowline — will determine whether you can form an Earth-like planet or something quite different. Although water makes up almost three-quarters of Earth’s surface area, it constitutes just a fraction of a percent of its mass. “This tells us that Earth formed interior to the snowline,” says Cleeves. “If it had formed outside the water snowline, we’d be a water world.”
Massive mapping
ALMA is also revealing much more about the composition of protoplanetary disks themselves, detecting several molecules that have never been seen before. In 2015, Öberg and her colleagues identified several types of complex cyanide molecules in the outer regions of the disk around the star MWC 480. Such molecules are thought to have been important for amino acid synthesis on Earth, and because they exist in comets in our solar system, researchers have speculated that comets may have carried them to our planet. The MWC 480 findings suggest that such a scenario might extend to other star systems, too.
But perhaps most exciting are the results that are still on the horizon. A massive international project called Molecules with ALMA at Planet-forming Scales — MAPS for short — led by Öberg, Bergin, Walsh, Yuri Aikawa at the University of Tokyo, and Viviana Veloso Guzmán at Pontificia Universidad Católica in Chile, is surveying in detail the abundance and distribution of 20 molecules in the gas of five protoplanetary disks. Through stunning sets of images, that work is revealing how similarities and differences in the elemental composition of the disks might contribute to the final makeup of molecules on a planet. For example, nitriles — molecules with a carbon triple bond to nitrogen, which are thought to be feedstock molecules for prebiotic chemistry — appear to be concentrated in the inner planet-forming regions of disks, whereas other molecules show a different distribution.
The full findings will be published later this year as part of a 20-paper stand-alone issue of The Astrophysical Journal. “Karin was really the driving force bringing the team together,” says Walsh. What’s more, most of the MAPS subprojects are led by early-stage researchers, providing them with the opportunity to conduct career-launching work, Walsh adds. “I think that’s a really good thing for the field.”
The data from MAPS have yielded some surprises. “One big thing we are starting to realize is that the elemental composition of the gas is very different from what we thought it was,” Öberg says. Most significantly, it contains much less oxygen than researchers expected — an important finding because oxygen abundance is so crucial for establishing planetary chemistry. What this means for planet formation remains to be determined, but if planets generally form with an oxygen-poor atmosphere this could be good news for origins of life researchers, since most origins-of-life chemistry scenarios rely on the presence of cyanides and related molecules, which are much easier to form in oxygen-poor environments.
Although researchers have not figured out exactly how life formed on Earth or detected it elsewhere, Öberg says, the research so far on protoplanetary and young-Earth chemistry, and the bounty of exoplanets discovered to date, makes her “cautiously optimistic” about the possibility. “The things we are pretty certain we need — a temperate rocky planet with access to water and organics — seem fairly common.”
But so far, with in-depth observations of just a few disks, there’s not enough data to characterize a “typical” planet-forming environment or to understand how much variation to expect in the composition of the planets they yield. Further observations of different types of molecules in a higher number of disks will be key to filling out the picture. “We have only scratched the surface of what ALMA can do,” says Bergin.
Researchers are also fired up about the James Webb Space Telescope (JWST), set to launch later this year, which promises to reveal mysteries that ALMA can’t solve. JWST’s power lies in its ability to detect near-infrared and infrared wavelengths, enabling it to probe the chemistry of the protoplanetary disk’s inner 5 AU — which ALMA can’t read. Öberg’s group already has a project approved to observe the same five MAPS disks with JWST. Additionally, related projects will provide a readout of molecules in the ice, which are also invisible to ALMA, but which play a crucial role in defining a planet’s chemistry. Combining the next round of ALMA data with JWST’s will hopefully reveal a more complete picture of the chemistry in the disk’s outer realm. This should yield crucial information about which organic molecules might be delivered to terrestrial planets through comet or asteroid impact.
Many of today’s insights into the chemistry of planet formation have been largely descriptive, which doesn’t give researchers the full story. “We have far too little quantitative data on the kinds of chemical reactions that can happen in these extreme environments,” Öberg says.
But that stands to change soon. By triangulating the next generation of observations with laboratory studies and computational modeling of chemical reactions under different conditions, researchers may be able to pin down the link between the detailed distribution of molecules and a planet’s molecular makeup at all stages of growth — from protoplanetary disk to fully formed exoplanet. “That is the end goal, though I think we are a few generations away from that,” Öberg says. “My job is to put down a few pieces of the puzzle — and then to help raise a generation that’s coming after me that can put down more pieces.”