Unveiling the Forces Behind Millions of Years of Cell Division
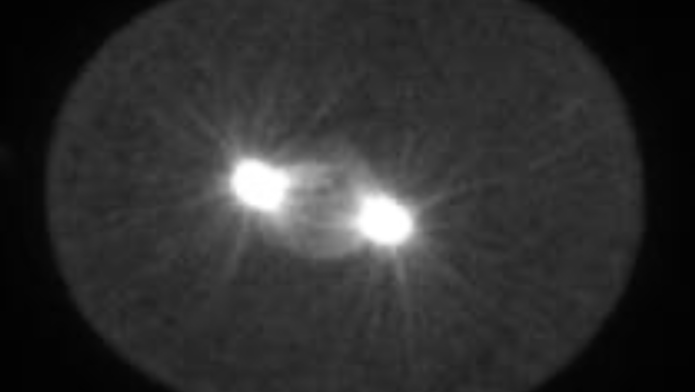
By clicking to watch this video, you agree to our privacy policy.
The human body is composed of more than 1 trillion cells, yet each human body begins as just one cell. To go from one to 1 trillion requires cell division: the very precise process by which chromosomes, the cell’s genetic material, are brought together at the cell’s center and then swiftly separated, as one cell becomes two. When the process is viewed under a microscope, ‘threads’ appear to pull the chromosomes apart in a graceful motion — like an invisible puppeteer pulling strings. These so-called threads directing the choreography of separation are part of the spindle, itself a complex of microscopic structures that lies at the heart of cell division. Interestingly, the spindle has been observed to vary in form in concert with the changing cell-division machinery during development and, more broadly, throughout evolution. However, despite the spindle’s ubiquity in biology, the nature of the forces acting on it has been a persistent scientific mystery.
Now, a study from a team at the Flatiron Institute’s Center for Computational Biology (CCB) has shed new light on the intricacies of spindle behavior. The study, published on September 23 in eLife, analyzed genetic and cellular data from roundworm populations to develop a mechanistic model that goes beyond biology to employ techniques from the related field of biophysics. The advantages of this new biophysical model lie in its ability to account for the variation in spindle traits like length and position that have been observed, for example, in roundworm populations separated by 100 million years of evolution. The approach taken here may also be applied to other long-standing mysteries within the cell, laying the foundation for a deeper, more robust understanding of how distinct regions within the cell become integrated, scaled and positioned across evolutionary time.
“Most evolutionary biology is approached mainly through genetics, not physics,” says Reza Farhadifar, a research scientist at CCB and first author of the paper. “Here, having a biophysical model to explain evolutionary processes is very novel. Through our approach, we can [now] understand evolution at the cellular level.”
The spindle is primarily made up of microscopic, hairlike proteins called microtubules that emanate from structures inside the cell called centrosomes. Just prior to cell division, the centrosomes migrate to opposite sides of the cell. The distance between these centrosomes defines spindle length. Motor proteins that drive microtubule motion are also part of the spindle. These components all work together, following a precise choreography to drive cell division and ensuring that future generations of cells will repeat the process.
For researchers delving into the mechanics of cell division and size, the roundworm makes an ideal model system. The roundworm’s first round of cell division is asymmetric, meaning that it produces two different-size cells. In biology, asymmetry is essential. It enables embryos to develop differentiated segments, such as a head and a tail. By examining known evolutionary connections between the many different roundworm species, scientists can study the variation in how the sizes of cellular components, including the spindle, scale with cell size in cell division. “How cells regulate the size of internal structures is a big topic in cell biology,” says Michael Shelley, director of CCB and one of the lead investigators on the paper.
In the eLife study, the researchers analyzed 12 million images from high-throughput microscopy of cell division in a panel of genetically diverse roundworms. They first quantified the variation they saw in the spindle during anaphase (the part of cell division when the chromosomes move to opposite sides of the cell), noting features such as initial and final spindle length, how quickly the centrosomes moved to their final position, and overall cell size. With this catalog of variation in hand, they then tested different types, or classes, of models that scientists have proposed to explain how the spindle elongates and stabilizes prior to cell division.
One such class, called Boundary models, posits that the final spindle length increases as the cell grows in size and decreases as centrosomes move away from the cell periphery, like a rubber band being stretched between two fingers. This model assumes no correlation between centrosome distance and cell length. The catalog showed that while final spindle length did increase with cell length — as the Boundary model predicts — centrosome distance also increased with cell length, in direct conflict with the expectations of the Boundary model. In fact, as the researchers evaluated other classes of models, a consistent relationship between cell length and final spindle length stood out. “The length of the cell is critical,” says Farhadifar. Indeed, previous work by Farhadifar and others showed how natural selection, by acting on cell length specifically, affects the roundworm spindle. “It’s remarkable that the single feature of cell length impacts so much about the cell,” adds Shelley.
To probe the forces at work in more detail, the researchers then performed laser ablation within the roundworm cells. Like a pair of microscopic scissors, laser ablation can sever astral microtubules, a type of microtubule that radiates out from the centrosomes. The research team could then see how this affected the centrosomes’ movement and final positions within roundworm cells during division. “There was an idea among scientists that pulling forces [alone] are intrinsically destabilizing,” says Daniel Needleman, a senior research scientist at CCB, a professor of applied physics and molecular and cellular biology at Harvard University, and co-lead investigator of the work. However, rather than finding a combination of pulling and pushing forces, as some scientists expected, they found that pulling forces, acting on the astral microtubules in different directions, gave stability to the centrosomes’ final positions. “With better measurements, things are revealed that may not have occurred to you or anyone else,” says Needleman.
Based on the experimental results, the researchers came up with a model that showed how directionally different pulling forces, resulting from one motor protein binding to one astral microtubule, can be stabilizing. Indeed, without this one-to-one constraint, the centrosomes’ final positions were unstable. Specifically, the model revealed that, if a microtubule from one centrosome binds to a motor protein anchored to the cell periphery, that protein is no longer available to any other microtubule from either centrosome. This creates a process akin to musical chairs between the two centrosomes that ultimately determines their positions within the cell. Importantly, the team’s model accounted not only for the catalog of variation generated in this study, but also for the variation in spindles characterized in previous work led by Farhadifar that examined roundworms’ 100-million-year evolutionary history.
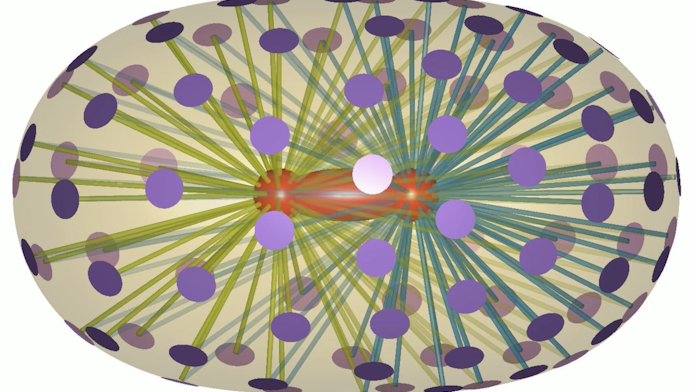
By clicking to watch this video, you agree to our privacy policy.
While understanding spindle dynamics is crucial in its own right — problems with cell division are responsible for cancers and infertility, for example — this work also has implications for other structures in the cell, says Farhadifar. “The approach we used in this paper combines evolutionary genetics, biophysics and mathematical modeling,” he says. “Taken together, these complementary approaches can deepen our understanding of size regulation and scaling of other cellular organelles such as the nucleus.”
“This is a rare instance of a biophysical model shining light on a fundamental determinant — cell length — of how things are scaled in a cell,” says Shelley.
In addition to its scientific contributions, this work highlights the importance of cross-disciplinary teams. For this study, researchers from the Faculty of Medicine Carl Gustav Carus in Dresden, biologists at New York University, biophysicists at Harvard University, and computational and modeling experts at the Flatiron Institute brought different perspectives and approaches to a singular problem. Long-term, uninterrupted collaborations like these that combine theory and experiments and are informed by enormous datasets are a hallmark of research at the Flatiron Institute. “At CCB, we are examining fundamental processes in cell biology with mathematical models, simulations and experiments,” says Shelley.
“Like the people who use fluid dynamics to study birds in flight, we are using physical principles to understand subcellular processes,” says Needleman.