Hilbert’s Problems: 23 and Math
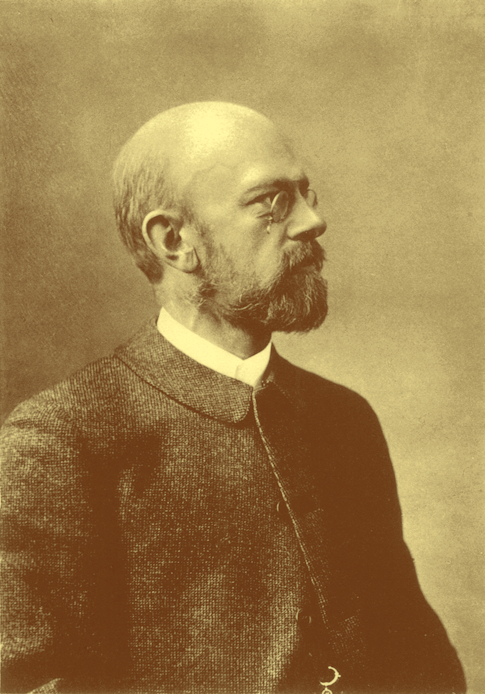
At a conference in Paris in 1900, the German mathematician David Hilbert presented a list of unsolved problems in mathematics. He ultimately put forth 23 problems that to some extent set the research agenda for mathematics in the 20th century. In the 120 years since Hilbert’s talk, some of his problems, typically referred to by number, have been solved and some are still open, but most important, they have spurred innovation and generalization. The Clay Mathematics Institute’s Millennium Prizes are a 21st-century version of Hilbert’s original proposal.
? = UNRESOLVED
1. CONTINUUM HYPOTHESIS. To mathematicians, all infinities are not the same. The infinity of the counting numbers — 1, 2, 3, … — is smaller than the infinity of all the real numbers. And there are towers of still greater infinities beyond the reals. Hilbert’s first problem, also known as the continuum hypothesis, is the statement that there is no infinity in between the infinity of the counting numbers and the infinity of the real numbers. In 1940, Kurt Gödel showed that the continuum hypothesis cannot be proved using the standard axioms of mathematics. In 1963, Paul Cohen showed it cannot be disproved, making the continuum hypothesis independent of the axioms of mathematics.
? 2.THE COMPATIBILITY OF THE STANDARD AXIOMS OF ARITHMETIC. Hilbert’s second problem was to prove that arithmetic is consistent, that is, that no contradictions arise from the basic assumptions he had put forth in one of his papers. This problem has been partially resolved in the negative: Kurt Gödel showed with his incompleteness theorems in 1931 that it is impossible to prove the consistency of a system called Peano arithmetic using only the axioms of Peano arithmetic. Mathematicians debate whether Gödel’s work is a satisfying resolution to the problem.
3. EQUIDECOMPOSABILITY. Any polygon can be cut into a finite number of polygonal pieces and reassembled into the shape of any other polygon with the same area. Hilbert’s third problem — the first to be resolved — is whether the same holds for three-dimensional polyhedra. Hilbert’s student Max Dehn answered the question in the negative, showing that a cube cannot be cut into a finite number of polyhedral pieces and reassembled into a tetrahedron of the same volume.
4. THE STRAIGHT LINE AS THE SHORTEST DISTANCE BETWEEN POINTS. Hilbert’s fourth problem is about what happens when you relax the rules of Euclidean geometry. Specifically, what geometries can exist in which a straight line is the shortest distance between two points but in which some axioms of Euclidean geometry are abandoned? Some mathematicians consider the problem too vague to have a real resolution, but there are solutions for some interpretations of the question.
5. UNDERSTANDING LIE GROUPS. Hilbert’s fifth problem concerns Lie groups, which are algebraic objects that describe continuous transformations. Hilbert’s question is whether Lie’s original framework, which assumes that certain functions are differentiable, works without the assumption of differentiability. In 1952, Andrew Gleason, Deane Montgomery and Leo Zippin answered the question, showing that the same theory arises whether differentiability is assumed or not. Some mathematicians have interpreted the question differently and consequently have different answers.
? 6.THE AXIOMATIZATION OF PHYSICS. One of Hilbert’s primary concerns was to understand the foundations of mathematics and, if none existed, to develop rigorous foundations by reducing a system to its basic truths, or axioms. Hilbert’s sixth problem is to extend that axiomatization to branches of physics that are highly mathematical. Some progress has been made in placing some fields of physics on axiomatic foundations, but because there is no ‘theory of everything’ in physics yet, a general axiomatization has not occurred.
7. IRRATIONALITY AND TRANSCENDENCE OF CERTAIN NUMBERS.
A number is called algebraic if it can be the zero of a polynomial with rational coefficients. For example, 2 is a zero of the polynomial x − 2, and √2 is a zero of the polynomial x2 − 2. Algebraic numbers can be either rational or irrational; transcendental numbers like π are irrational numbers that are not algebraic. Hilbert’s seventh problem concerns powers of algebraic numbers. Consider the expression ab, where a is an algebraic number other than 0 or 1 and b is an irrational algebraic number. Must ab be transcendental? In 1934, Aleksandr Gelfond showed that the answer is yes.
? 8.PROBLEMS OF PRIME NUMBERS. Hilbert’s eighth problem includes the famous Riemann hypothesis, along with some other questions about prime numbers.
? 9.RECIPROCITY LAWS AND ALGEBRAIC NUMBER FIELDS. Hilbert’s ninth problem is on algebraic number fields, extensions of the rational numbers to include, say, √2 or certain complex numbers. Hilbert asked for the most general form of a reciprocity law in any algebraic number field, that is, the conditions that determine which polynomials can be solved within the number field. Partial solutions by Emil Artin, Teiji Takagi and Helmut Hasse have pushed the field further, although the question has not been answered in full. The closely related 12th problem, which deals with other extensions of the rational numbers, is unresolved.
10. SOLVABILITY OF A DIOPHANTINE EQUATION. Polynomial equations in a finite number of variables with integer coefficients are known as Diophantine equations. Equations like x2 − y3 = 7 and x2 + y2 = z2 are examples. For centuries, mathematicians have wondered whether certain Diophantine equations have integer solutions. Hilbert’s 10th problem asks whether there is an algorithm to determine whether a given Diophantine equation has integer solutions or not. In 1970, Yuri Matiyasevich completed a proof that no such algorithm exists.
? 11.ARBITRARY QUADRATIC FORMS. Hilbert’s 11th problem also concerns algebraic number fields. A quadratic form is an expression, like x2 + 2xy + y2, with integer coefficients in which each term has unknowns raised to a total degree of 2. The number 9 can be represented using integers in the above quadratic form — set x equal to 1 and y equal to 2 — but the number 8 cannot be represented by integers in that quadratic form. Some different quadratic forms can represent the same sets of whole numbers. Hilbert asked for a way to classify quadratic forms to determine whether two forms represent the same set of numbers. Some progress has been made, but the question is unresolved.
? 12.EXTENSION OF KRONEKER’S THEOREM ON ABELIAN FIELDS TO ANY ALGEBRAIC REALM OF RATIONALITY. With his 12th problem, Hilbert sought to generalize a theorem about the structure of certain extensions of the rational numbers to other number fields. It is currently unresolved.
13. SEVENTH-DEGREE POLYNOMIALS. Hilbert’s 13th problem is about equations of the form x7 + ax3 + bx2 + cx + 1 = 0. He asked whether solutions to these functions can be written as the composition of finitely many two-variable functions. (Hilbert believed they could not be.) In 1957, Andrey Kolmogorov and Vladimir Arnold proved that each continuous function of n variables — including the case in which n = 7 — can be written as a composition of continuous functions of two variables. However, if stricter conditions than mere continuity are imposed on the functions, the question is still open.
14. FINITENESS OF CERTAIN SYSTEMS OF FUNCTIONS. The motivation for Hilbert’s 14th problem came from previous work he had done showing that algebraic structures called rings arising in a particular way from larger structures must be finitely generated; that is, they could be described using only a finite number of building blocks. Hilbert asked whether the same was true for a broader class of rings. In 1958 Masayoshi Nagata resolved the question by finding a counterexample.
? 15.RIGOROUS FOUNDATION OF SCHUBERT’S ENUMERATIVE CALCULUS. Hilbert’s 15th problem is another question of rigor. He called for mathematicians to put Schubert’s enumerative calculus, a branch of mathematics dealing with counting problems in geometry, on a rigorous footing. Mathematicians have come a long way on this, though the problem is not completely resolved.
? 16.TOPOLOGY OF ALGEBRAIC CURVES AND SURFACES. Hilbert’s 16th problem is an expansion of grade school graphing questions. An equation of the form ax + by = c is a line; an equation with squared terms is a conic section of some form — parabola, ellipse or hyperbola. Hilbert sought a more general theory of the shapes that higher-degree polynomials could have. So far the question is unresolved, even for polynomials with the relatively small degree of 8.
17. EXPRESSION OF DEFINITE FORMS BY SQUARES. Some polynomials with inputs in the real numbers always take non-negative values; an easy example is x2 + y2. Hilbert’s 17th problem asks whether such a polynomial can always be written as the sum of squares of rational functions (a rational function is the quotient of two polynomials). In 1927, Emil Artin solved the question in the affirmative.
18. BUILDING UP OF SPACE FROM CONGRUENT POLYHEDRA. Hilbert’s 18th problem is a collection of several questions in Euclidean geometry. First, for each n, does Euclidean space of dimension n have only a finite number of fundamentally distinct translation-invariant symmetries? In 1910, Ludwig Bieberbach answered this part of the question in the affirmative. Second, in a tiling of the plane by squares, any square can be mapped to any other square. Such a tiling, in any dimension, is called isohedral. This part of the problem concerns the existence of non-isohedral tilings in three-dimensional space. In 1928, Karl Reinhardt found such a tiling. (Later, Heinrich Heesch found a tiling in two-dimensional space; though Hilbert did not say why he did not ask the same question about two-dimensional space; many people assume it is because he did not realize such a tiling could exist there.) Finally, what is the densest way to pack spheres? In 1998, Thomas Hales presented a computer-aided proof showing that the typical configuration in produce stands is indeed optimal.
19 and 20. ARE THE SOLUTIONS OF REGULAR PROBLEMS IN THE CALCULUS OF VARIATIONS ALWAYS NECESSARILY ANALYTIC? Do solutions in general exist? The calculus of variations is a field concerned with optimizing certain types of functions called functionals. In his 19th and 20th problems, Hilbert asked whether certain classes of problems in the calculus of variations have solutions (his 20th) and, if so, whether those solutions are particularly smooth (19th).
21. LINEAR DIFFERENTIAL EQUATIONS WITH PRESCRIBED MONODROMY. Hilbert’s 21st problem is about the existence of certain systems of differential equations with given singular points and the systems’ behavior around those points, called monodromy. Josip Plemelj published what was believed to be a solution in 1908, though much later Andrei Bolibrukh found a counterexample to Plemelj’s work, showing that such systems of equations do not have to exist.
? 22. UNIFORMIZATION. Hilbert’s 22nd problem asks whether every algebraic or analytic curve — solutions to polynomial equations — can be written in terms of single-valued functions. The problem has been resolved in the one-dimensional case and continues to be studied in other cases.
? 23. FURTHER DEVELOPMENTS IN THE CALCULUS OF VARIATIONS. The calculus of variations has undergone robust development — including the solutions to the 19th and 20th problems — in the 120 years since Hilbert posed these questions. But Hilbert’s phrasing does not specifically indicate a clear endpoint, so this ‘problem’ can never be considered resolved per se.