
New Efforts to Relate Brain Structure and Function Reveal Surprising Properties of Neural Circuits
Nematodes don’t like to be touched. A poke will send the worm slithering backward, away from the bothersome nudge. Unless, that is, the worm was in the midst of turning when it was poked. “Somehow, the act of turning makes the worm change the way it responds to this very salient stimulus, but we didn’t know how this computation played out in the brain,” says Andrew Leifer, a neuroscientist at Princeton University and an investigator with the Simons Collaboration on the Global Brain.
Despite having a complete connectome mapping every neuron and synapse in the Caenorhabditis elegans brain, Leifer and collaborators still struggled to understand how the worm’s touch and turning systems interact. “As you start to trace where these circuits meet, you end up in this dense web of anatomical connections, and it’s not clear which paths are most relevant or how signals are moving through them,” Leifer says.
Over time, the team found themselves reaching a similar limit for each circuit they explored. “We eventually realized everyone in the field faces this same problem: The connectome shows you putative circuit mechanisms, but observing how information actually flows through the network is much less straightforward,” Leifer says. “We finally thought: Why don’t we just measure all functional connections across the brain and provide a resource that could be used similarly to how the structural connectome has been used?”
Led by postdoc Francesco Randi, the team launched a massive effort to optogenetically activate each of the 302 neurons in the C. elegans brain while simultaneously imaging the responses of all other neurons. The resulting functional connectivity atlas, posted on the arXiv in August, describes functional connections across more than 10,000 neuronal pairs — many of which were observed for the first time.
One of these never-before-seen connections happened to be between neurons from the turning and touch circuits. It turns out that one neuron inhibits the other, even though there is no direct synapse between them. Rather, this inhibition is the result of an indirect or ‘effective’ connection, invisible on the anatomical map. “Before this experiment we didn’t even know this connection existed, and now it’s informing our understanding of movement coordination,” Leifer says. “We’re now excited to see what other circuit computations can be explained through functional connectomics.”
These experiments are part of a growing effort to study neural circuits through the integration of structural and functional connectivity measures. The term ‘functional connectivity’ is commonly used in human neuroimaging to describe correlations in activity across brain areas, but modern imaging tools are now allowing researchers to make similar measurements in model organisms at single-cell resolution. In studies based on this traditional definition, correlated neuronal activity indicates a functional interaction, and statistical methods can help estimate which neuron is affecting which. In an even newer approach, studies like Leifer’s leverage advances in optogenetics to probe the directionality of neuronal connections more directly. By activating individual cells and imaging how the others respond, these studies measure what is increasingly referred to as ‘causal connectivity’ between neurons. Other groups are starting to combine these new large-scale anatomical and activity datasets to test specific hypotheses about brain areas and behaviors. Though the work is still in early days, the findings are highlighting important network properties in the worm, fly and mouse brains, and illuminating the complex relationship between circuit structure and function.
The promise of the worm
C. elegans is often thought to be an ideal system for understanding how structure gives rise to function. In 1986, its connectome became the first to be fully mapped, a massive achievement that’s informed countless discoveries on worm behaviors ranging from sensation and locomotion to feeding and reproduction. Still, the wiring diagram on its own is insufficient to fully illuminate the animal’s more complex behaviors, which tend to rely on more intricate neural networks. Here, broadscale functional tools provide a new layer of information that can reveal previously hidden aspects of the neural circuitry. “The technology is finally catching up to the promise of the worm,” Leifer says. “We’ve had the anatomy done for decades, but only now are we able to do these functional measurements at brain scale and cellular resolution.”

The ‘perturbation map’ produced by Leifer and collaborators represents a major technical advance that relied on several new techniques. To build the map, the researchers had to stimulate an individual neuron deep in the C. elegans brain while simultaneously imaging all others at high speed. This necessitated a complex optical setup pairing two-photon stimulation with single-photon spinning disk confocal microscopy. It also required an unconventional optogenetic protein that could be activated by purple light but wouldn’t respond to the blue light used to image the other neurons’ calcium response. “We spent quite a long time looking for a pair of proteins that would play nicely together,” Leifer says. Finally, they did all these experiments in a new multicolor worm strain that fluorescently labels each neuron with a distinct color, so cells are easier to identify when their functional activity is registered to the structural connectome.
One of the most striking findings to emerge from these experiments is a form of functional connectivity undetectable in the anatomical maps: extrasynaptic communication. Dense vesicles containing neuropeptides, monoamines or other neurotransmitters are released from parts of the neuron outside the synapse, where they then diffuse through the extracellular space onto receptors on surrounding cells. While these signals had been previously observed, they weren’t expected to play a major role in neural dynamics. The new findings indicate that they’re actually quite prevalent throughout the worm brain and contribute significantly to short-term neuronal activity, which implicates them in a wider range of behaviors than previously thought.
Such ‘wireless’ signaling is just one way that functional connections differ from what the neuroanatomy predicts, Leifer says. “But we’re also learning what features make structure and function more similar or more different.” For example, in a subnetwork of neurons in the worm pharynx that shows less extrasynaptic signaling, researchers saw better agreement between circuit structure and function.
A second worm study led by SCGB investigator Manuel Zimmer, a neuroscientist at the University of Vienna, used a more classic approach to describe functional connections in C. elegans. Here, researchers imaged spontaneous activity across the worm’s brain and then measured the correlation between each neuron pair. They then looked to see if the structural connectome could predict the functional connectivity patterns.
The results, published in July 2022 in Current Biology, revealed surprising discrepancies. Chemical synapses were not particularly good indicators of functional connectivity in the worm, meaning two directly connected neurons are not consistently active at the same time. The presence of electrical synapses, in which ions flow between neurons through gap junctions, was a better predictor, but not by much. Taking synaptic strength into account also didn’t help the predictive power of the anatomical connectome. “For a pair of neurons, it only mattered if they were connected or not; the number of synapses between them didn’t make a difference,” Zimmer says.
In fact, the functional connectivity of each neuron pair was mostly predicted by nonlocal circuit features, such as whether or not they had a common input. “Two neurons on opposite sides of the brain that have no anatomical connection but receive similar inputs will often show more correlated activity than those that are directly connected to each other,” Zimmer says.
Both studies highlight the importance of combining structural and functional information, and they offer complementary approaches to measuring functional connectivity. Spontaneous activity may more closely reflect how the neurons normally behave, while systematically activating every neuron ensures no part of the circuit goes unexplored.
Function in the fly
Compared to the worm brain, the fruit fly brain, with its roughly 100,000 neurons, provides researchers with a more complex web of connections and richer behavioral repertoire to explore. Thanks to recent advances in fly connectomics, they can now investigate both at broader scales and at finer resolution. Mala Murthy, a neuroscientist at Princeton and an SCGB investigator, is part of the scientific community advancing whole-brain connectomics in the fly. Her latest project, a collaboration with Sebastian Seung called FlyWire, allows neuroscientists to crowdsource and therefore speed up the process of proofreading neural reconstructions, the final step to completing a connectome (for more, see “The Connected Connectome”). Murthy and colleagues are now leveraging these comprehensive reconstructions to explore neural dynamics in the fly, focusing on how the structure of sensory circuits gives rise to neurons’ feature selectivity.
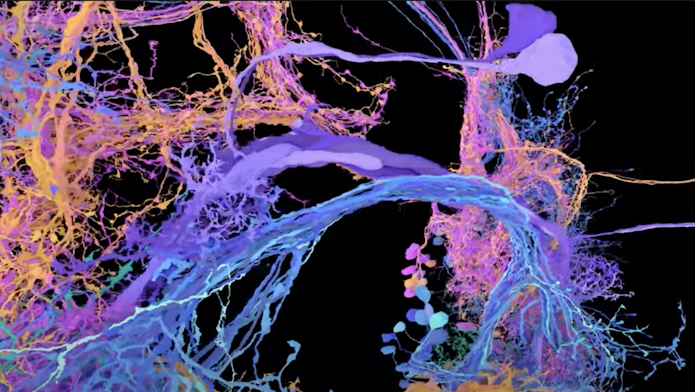
By clicking to watch this video, you agree to our privacy policy.
One such effort, published in July 2022 in Current Biology, focused on the female fly auditory circuit. A main function of this circuit is to help coordinate an appropriate response to the male courtship song, which alternates between two main modes — sine and pulse. Prior work suggested that the auditory circuit might be designed to separate sine and pulse signals into two separate processing streams.
To test this hypothesis, postdoc Christa Baker first determined whether each auditory neuron preferred sine or pulse song, and then compared the results with the neurons’ anatomical connectivity in the FlyWire diagram. While some neurons did have a distinct preference for sine or pulse song, the team was surprised to find that many showed a mixed or intermediate preference. And rather than being segregated into distinct sine and pulse pathways, all these different neurons were actually densely interconnected with no obvious hierarchical structure among them. Researchers don’t yet know exactly why the circuit is designed this way, but their models suggest that this interconnectivity helps generate the tuning of the mixed-preference population. Furthermore, recurrence within the circuit may help the female fly process features of the courtship song over longer timescales or integrate the information with other sensory signals.
“It’s clear the fly auditory system is not just a simple feedforward sensory circuit, but instead contains a lot of recurrent connections, as well as interconnectivity with other brain areas,” says Murthy. “I think this surprises people because up until now, we’ve been studying these sensory pathways within a feedforward framework, but the fly brain is much more of a multisensory feedback control system, and the new structural and functional data really makes that clear.”
Functional connections have also been measured through perturbational approaches in the fly, though not at the whole-brain scale as in Leifer’s worm study. In a 2018 paper published in eLife, Vivek Jayaraman’s lab at the Janelia Research Campus used simultaneous optogenetic stimulation and calcium imaging to test the connectivity between 70 cell pairs in the fly brain. Comparing these causal connectivity measures with the anatomical connectome, Jayarman, Romain Franconville and collaborators found that functional connectivity appeared much sparser than anticipated — in other words, activating a neuron generated responses in far fewer downstream neurons than might be expected based on the connectome.
However, Jayaraman notes that their optogenetic protocol was designed to provide minimal stimulation, with the goal of only activating direct synaptic connections. This means additional functional connections at weaker synapses or those that require the coactivation of multiple presynaptic neurons may not be detected in these experimental conditions.
Still, Jayaraman says “it’s clear the anatomy is merely a scaffold for computation that the functional circuit is then laid on top of.” An engineer by training, Jayaraman has often relied on computational models to explore such neural network dynamics. “We have long been trying to relate structure to function through theory, and now we can test a lot of these ideas quite directly through functional connectomics.”
It’s not yet clear how specific lessons learned from the worm or fly will translate to other organisms, but these early findings suggest each species may adopt distinct mechanisms for optimizing its neural circuit’s processing capacity. “The match between structural and functional connectomes is going to vary across different species,” says Murthy. “Extrasynaptic connections may help the C. elegans brain build a larger repertoire of communication with fewer neurons, but the larger the nervous system, the more you can wire in this specificity with more cells.” Murthy and Jayaraman suggest it’s the circuit topology, rather than topography, that’s most likely to generalize across invertebrate and vertebrate species. Physical maps and mechanisms may differ, but the resulting functions and computations of the networks may be similar. “The question is: How does complex connectivity give rise to these population signals and behaviors?” says Murthy. “We’re just scratching the surface of that right now, but this is the fun we’re going to have in the next few years.”
Mapping the mouse brain
Invertebrate models, with their small brains and sparse circuits, have paved the way in structural connectomics. But when it comes to measuring functional connectivity, mouse labs are also making great strides. In a new study from SCGB investigator Karel Svoboda’s lab at the Janelia Research Campus, Arseny Finkelstein, now at Tel Aviv University, built on recent technical advances to measure causal connectivity in the mouse motor cortex. Through the high-speed activation and imaging of individual neurons, Finkelstein was able to map connections between half a million neuron pairs within a 30-minute session — a tenfold scale-up from previous mouse studies.
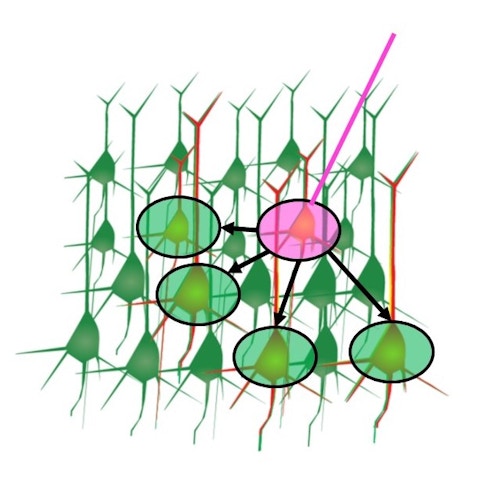
Using this strategy, Finkelstein explored the functional organization of the untrained motor cortex. “Much of what we know about the motor cortex comes from animals expertly trained on a specific task,” he says, “but what do these circuits look like before learning occurs?” To answer this, Finkelstein focused on the anterior lateral motor cortex (ALM), an area involved in coordinating mouse lick movements. He first had naïve mice perform a simple lick task to determine each neuron’s preferred lick direction; then he stimulated each neuron while measuring how the others responded.
The results, presented at the Cosyne meeting in Lisbon in March, revealed a high level of functional organization in the ALM. Similarly tuned neurons appeared to be grouped in cortical mini-columns where they excited each other but inhibited differently tuned neurons just outside the column. This motif has been seen in primate primary sensory areas and to some extent in fly brains as well, but it was less established in these higher-order frontal cortices.
Another major finding was the presence of hub neurons, cells with dense outgoing connections, weaker feature selectivity, and more reliable firing patterns. Researchers don’t yet know exactly what these hub neurons are doing, but they speculate that the cells are placed throughout the circuit to help organize brainwide activity. “We think that rather than representing a specific feature of the motor plan, these hub neurons may work to initiate motor sequences and orchestrate broader cortical dynamics,” Svoboda says.
Zimmer’s C. elegans study also identified hub neurons, highlighting a potentially conserved circuit motif for coordinating brainwide activity patterns. In worms, sharing a connection with one of these hub neurons was a major predictor of functional connectivity between neurons. Indeed, inhibiting a few of these hub neurons was enough to disrupt brainwide correlations in the worm. As functional connectivity studies continue to reveal network properties in each model system, scientists are interested to see what other circuit motifs are conserved across species or specialized in each.
One of the biggest open questions — and the most challenging to answer — is how stable these connectomes are in each organism across individuals and timescales. Fly and worm researchers will likely be the first to address this, as methods for imaging, reconstructing and proofreading their connectomes become more streamlined. Murthy, for example, is interested in comparing structure and function across individual flies. “The relative stereotypy of the fly brain is a huge advantage for neuroscientists, but there may still be some interesting sources of variability that would be exciting to understand and relate to function.”
Vertebrates’ larger and more complex brains are likely to be much more variable. “Mammalian systems are specialized for learning and flexibility, so we can expect to see a lot more variability in their structural and functional connections, as well as circuit motifs designed to support that sort of flexibility,” says Svoboda, who recently moved from Janelia to the Allen Institute in Seattle, where he is now studying whole-brain connectivity. He estimates we’re at least a decade away from having a full mouse connectome, though some mouse researchers are starting to collect retrospective reconstructions of smaller brain volumes after they’ve been functionally imaged.
Researchers eventually hope to ask how functional connectivity changes not just from species to species or from individual to individual, but across learning and brain states. It’s why Finkelstein chose to do his functional connectivity measures in naïve, untrained mice. “Having this snapshot of ‘baseline’ functional connectivity will allow me to one day ask questions about how the circuit can be modified,” he says. “But there’s still a lot of questions to answer on the way there.”